
Old Drugs Offer a New Route for Exploring Neural Dynamics
Though humans have been exploring psychoactive drugs since ancient times, how they actually work remains largely a mystery. Researchers have made extensive progress unpacking the biochemical impacts of different drugs, such as their target molecules and how they shape synaptic transmission. But uncovering how specific drugs alter neural activity to enhance attention, alleviate depression or dampen tremors remains a much more difficult challenge.
This is beginning to change, though, as researchers employ the tools of systems and computational neuroscience to revisit pharmacology with a fresh perspective. These scientists hope exploring how specific compounds alter neural dynamics will offer both new insight into how these drugs exert their cognitive effects and a novel means for testing basic hypotheses of neural coding.
“Psychiatric drugs hold tremendous potential for probing complex or cognitive functions like attention, memory, decision-making and movement, but it’s only been very recently that we’ve started to formulate solid hypotheses about how these processes work at the systems and computational levels,” says Nicole Rust, a neuroscientist at University of Pennsylvania and an investigator with the Simons Collaboration on the Global Brain. Rust is excited about what she calls this “vintage-modern” approach of combining old drugs with highly computational hypotheses.
These new developments are unfolding amid an explosion in the availability of tools for recording activity in larger populations of neurons with single cell resolution, along with advances in data analysis and theory. The result is a slew of newly identified correlations between neural activity and complex behaviors, along with new models that attempt to explain them. Now scientists are looking for ways to manipulate these systems and put their models to the test.

Traditional methods for perturbing neural activity, such as lesions, offer a powerful hammer approach, but they may not always be focused enough or physiologically relevant. Modern tools like opto- or chemogenetics offer higher spatial and temporal resolution, but don’t always yield clear effects on behavior. For many higher-order cognitive processes, there may not even be obvious brain regions, projections or cell types to target. Psychoactive drugs, delivered systemically, offer a way to tinker with complex processes that are distributed across the brain. Researchers can select a drug based on its known behavioral effects and use that to test hypotheses about the underlying neurophysiology. The hope is that the results will provide both basic and clinical insights, helping systems neuroscience become more easily translatable.
“A lot of these drugs were discovered before we even knew anything about neurotransmission, but they may now be the key to answering many of our questions about neural activity,” Rust says.
Shifting signatures with Ritalin
Methylphenidate, known commercially as Ritalin, is a leading drug for treating Attention Deficit Hyperactivity Disorder (ADHD) in children and adults. At the molecular and cellular levels, Ritalin interacts with dopamine and norepinephrine systems and modulates synaptic plasticity. “But if someone asks us how these things help people pay attention, I don’t have an answer,” Rust says.
That’s beginning to change, thanks to recent work from the lab of Marlene Cohen, a neuroscientist at the University of Pittsburgh and an SCGB investigator. Cohen is a pioneer in computational models of visual perception and attention, informed by multielectrode recordings in rhesus macaques. In research published in PNAS in April 2022, Cohen and collaborators made major strides in explaining Ritalin’s mechanism of action. The choice to study the drug was motivated by more basic science questions. “We were mostly just looking for a perturbational tool that would have a good chance of changing behavior,” Cohen says.
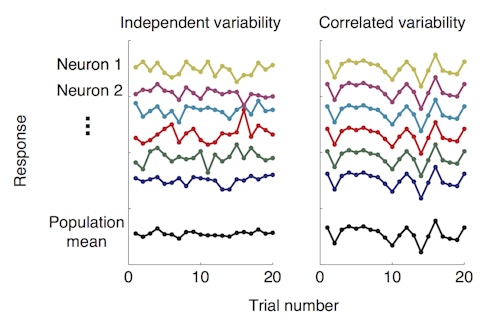
For years, her group had been training macaques to perform a spatial attention task while the group monitored activity in visual cortex with microelectrode arrays. In the task, monkeys are presented with two visual stimuli, cued to attend to one of them and asked to detect subtle changes in one or the other. Perhaps not surprisingly, they tend to perform better when the stimulus that changes is the one to which they were paying attention. In a previous study published in Science in January 2018, postdoctoral researcher and SCGB fellow Amy Ni found that while animals were paying attention to one of the visual stimuli, the neurons responding to that stimulus showed less correlated variability, a measure of how much the activity of individual neurons in a group tend to fluctuate together.
In other words, attention seemed to dampen the correlations among neurons, allowing each neuron to act more independently from its neighbors. This helped the population encode more visual information, enhancing the signal-to-noise ratio and allowing more valuable information to flow through the circuit.
Equipped with an elegant hypothesis of attention, Cohen now wanted to test it further. “In most experiments, we measure the brain under a limited set of conditions and then make a model of how we think it’s working,” she says. “We needed something to push the system into a different part of the operation to see if our model still holds up.” Attention is a distributed process, and thus a distributed manipulation seemed like the clearest starting point. And since the drug seems to tap into the brain’s natural mechanisms of attention, scientists can manipulate these networks without having to understand them fully. “That drugs affect the brain systemically is both their weakness and their strength,” Cohen says.
The monkeys received an oral dose of Ritalin on alternating days prior to performing the spatial attention task. Cohen believed if changes in correlated neuronal variability were responsible for changes in attention and behavior, any observed change in the monkeys’ behavior under the influence of Ritalin should be associated with a corresponding change in this neural signature.
Indeed, this was exactly what they found. Ritalin improved the monkeys’ ability to detect visual changes in the attended stimuli. On these same trials, visual neurons tuned to the attended stimulus location showed decreased correlated variability. Importantly, Ritalin did not affect correlated variability in neurons when the monkey was not paying attention to the stimulus in their receptive field. So even though the drug is delivered systemically, it boosts only the relevant signal. “Ritalin helped them perceive the thing they were paying attention to, but not the other stimuli they were trying to ignore,” Cohen says.
“What Marlene did is so important, because she’s one of the first to actually establish a mechanism of action for a psychiatric drug at the systems and computational level, filling in this crucial gap to understanding attention in healthy and impaired states,” Rust says.
Cohen assumes Ritalin produces similar effects on signal-to-noise in other brain regions as well, and new work from SCGB investigator Krishna Shenoy’s lab at Stanford seems to agree. Graduate students Jessica Verhein and Saurabh Vyas are using Ritalin to probe hypotheses of neural coding in the primate motor cortex. For example, Shenoy and collaborators have shown that before a monkey makes a move, populations of motor cortical neurons show “preparatory” activity tuned to the upcoming reach direction. If Ritalin does indeed improve the signal-to-noise ratio in the way Cohen’s work suggests, this preparatory activity may show cleaner tuning to the upcoming reach. In preliminary results, that’s exactly what they found.
Shenoy’s lab has also identified specific neural signatures linked to reaction time and movement speed, both of which are boosted by Ritalin. Milliseconds before the monkey reaches, researchers see a ramping of motor neuron activity that correlates with reaction time. Verhein and Vyas are finding that in the presence of Ritalin, this “trigger” signal shows steeper ramping, and the monkey’s reaction time decreases. Once the animal begins to reach, an “execution” signal arises in the form of distinct rotational dynamics in the population activity. However, it’s not yet clear how movement speed is represented in these rotations. The researchers are now finding that Ritalin produces faster reaching movements, which seems to translate to a higher frequency of rotations. “Ritalin has been a great tool for learning about these neural signatures and testing how they relate to movement,” Verhein says. “It’s also extremely exciting that we can now potentially use this information to develop more targeted therapies for improving motor function.”
Disconnecting with ketamine
While Ritalin seems to enhance existing cognitive mechanisms, researchers are turning to ketamine seeking the opposite — the drug’s unique ability to perturb cognition beyond recognition. Ketamine is an anesthetic medication that induces dissociation and out-of-body experiences. These effects make it a powerful tool for studying how the brain processes who and where you are.
“Dissociation is an interesting brain state in which cognitive processes that are typically fused together are suddenly separated,” says Karl Deisseroth, a neuroscientist and psychiatrist at Stanford. “Perception, action and your sense of self are no longer unitary but instead competing streams of information, so in that way it’s a great window into some very fundamental neuroscience questions.”
In addition to drugs, dissociation can be caused by trauma, epilepsy or neuropsychiatric disorders. To study how this brain state affects neural activity, Deisseroth’s team gave mice ketamine and observed the entire dorsal cortex with wide-field imaging. Deisseroth says the “recent revolution” in brainwide imaging tools has been pivotal to exploring the systemic effects of psychiatric drugs. What they found was totally unexpected, he says.
Ketamine induced a 1 to 3 Hz rhythm focused in layer 5 of the retrosplenial cortex, which tracked with dissociative behavior. Oscillations in this frequency range are not new, but seeing them so localized to one cortical area was.
When researchers mimicked this pulsing through rhythmic optogenetic stimulation of retrosplenial neurons, they reproduced signs of dissociation in the mice. Motor reflexes showed the animals could still detect aversive stimuli, but they no longer displayed emotional or self-protective behaviors, suggesting a disconnect between sensory signals and the sense of self.
By recording from a corresponding brain area in a human epilepsy patient, researchers found that a similar rhythm emerged when the patient experienced pre-seizure dissociative auras. Scientists were even able to briefly electrically stimulate the brain region, which itself evoked dissociative sensations in the patient.
The researchers then went back to their mouse model to investigate how this localized rhythm contributed to dissociation. Using Neuropixel probes, they found that thalamic nuclei connected to the retrosplenial cortex received the oscillatory signal and fluctuated in sync. In turn, these nuclei inhibited other nearby nuclei connected to different cortical regions, desynchronizing these cortical networks from one another. “This was a striking and beautiful display of the role of thalamocortical modules to not only facilitate dissociation, but perhaps have an even more generalized role in gating which information is included or excluded in the current brain state,” Deisseroth says. The study was published in Nature in September 2020.
“The coolest thing about this study was that they recorded from a similar brain region in a human being and a mouse, and observed the effects of the same trigger in both,” says Rob Malenka, a neuroscientist and trained psychiatrist at Stanford who collaborated on the study. Malenka has long studied the molecular and cellular mechanisms of drugs, but he is hopeful that systems neuroscientists embracing pharmacology will glean new insights. “Neural dynamics are hard to interpret on their own, but having a drug that causes a distinct behavior gives you something to tie these interesting changes in neural activity to,” Malenka says.
Inspired by these insights into dissociation, other groups are now using ketamine to further explore spatial coding of the out-of-body experience. One such project is led by graduate student Francis Kei Masuda in the lab of Lisa Giocomo, a neuroscientist at Stanford and an SCGB investigator. Masuda is recording from grid cells in the medial entorhinal cortex while mice navigate a virtual reality hallway. Grid cells serve as the brain’s internal GPS system, representing an animal’s location in Cartesian space. In these experiments, each spatial cell represents a few different locations within the hallway, firing whenever the mouse passes through them.
“When someone is on ketamine, their sense of space is going haywire, so it was very tantalizing to see how that experience might be represented in the activity of these spatial cells,” Masuda says. Virtual reality mazes allow for some warping of spatial stimuli, but to manipulate the spatial experience to this extreme, “That’s not something that’s easy to re-create in any way other than with drugs,” he says.
Masuda starts the recordings before the ketamine kicks in, allowing him to watch the effects as the drug washes in and out of the mouse’s brain. Preliminary research shows that ketamine does alter spatial coding within the entorhinal cortex. Acutely, the cells no longer respond to spatial information, activating almost randomly with no reference to the mouse’s location.
Then, suddenly, as the drug wears off, the circuit snaps back together. Cells fire like grid cells again, but now they represent a different set of locations than they did before. Though the tuning of individual neurons has changed, the network as a whole still seems to compute and convey similar spatial information.
Masuda and colleagues are now digging into computational models of the circuit to explain how it can remap an environment so efficiently. He thinks something about this reset may help explain ketamine’s dissociative effects, and perhaps its clinical potential. “It’s not that you think you’re in a brand-new place when the ketamine wears off, but you might be seeing your surroundings with a fresh set of eyes,” Masuda says. “Perhaps this kind of refresh in the brain’s ongoing activity is related to ketamine’s positive effects on depression and rumination.”
These notions perhaps reflect the next chapter for this pharmacological approach. As Malenka suggests, “The biggest strength of drugs in systems neuroscience may be in the new questions they inspire.”