Expanding Neuroscience’s Menagerie of Model Animals
Neuroscience once housed a menagerie of creatures, each chosen for a special attribute. Squid have two giant axons, making them an ideal candidate for Alan Hodgkin and Andrew Huxley’s famous studies of electrical transmission. Sea slugs are capable of simple learning and have easily accessible neurons, providing a basis for seminal studies in learning and memory. Frogs’ large synapses offered a handy means of studying how neuronal signals are transmitted to muscle.
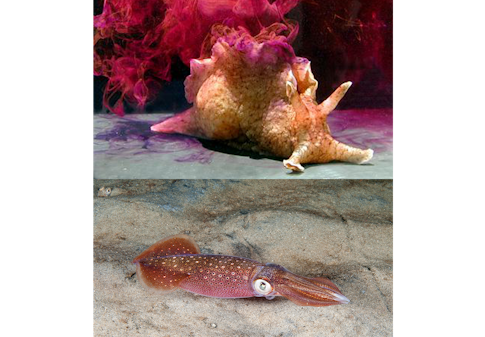
But the advent of molecular tools designed for specific species — particularly mice, flies and worms — dramatically shrank that pool. Most researchers gravitated toward species for which there was an existing arsenal of tools rather than developing new ones. “When I started in neuroscience 20 years ago, there was a diversity of species. There were frog labs and rabbit labs and crawfish labs,” says Michael Long, a neuroscientist at New York University and an investigator with the Simons Collaboration on the Global Brain (SCGB). “Now there is a mass consolidation of model systems, I think to the detriment of the field. Rather than being a jack-of-all-trades, these consensus models are often the master of none.”
Long and others are concerned that focusing on so few species makes it difficult to identify common principles in cognitive function — and features that are unique to a particular species. “The dominant model organisms have a great advantage: The knowledge base gives great power,” says Nachum Ulanovsky, a neuroscientist at the Weizmann Institute of Science in Israel who studies bats. “But maybe we are missing something. Does what we find in standard animal models, like the mouse, generalize? It’s important to study different species to understand what is invariant and what isn’t.”
For example, neuroscientists once theorized that continuous forms of theta oscillations, rhythmic waves of neural activity in the hippocampus, play an essential role in generating spatial firing patterns of navigational cells known as grid cells, based on research in rodents. But in 2011, Ulanovsky and his collaborator Michael Yartsev, now at the University of California, Berkeley, found that bats lack this form of oscillation, disproving that theory. Subsequent research showed the same is true for monkeys.
In a paper published in Cell last year, Ulanovsky’s team showed that although neurons in the bat hippocampus lack the oscillations seen in rodents, they do synchronize in a different way. “If we only studied mice, you would think oscillations are a general phenomenon, but they’re not,” Ulanovsky says. Synchronization, on the other hand, is present in both species and may be important for the function of the hippocampus.
“I think it is important if you want to understand something as complicated as the brain to have a reasonably good sampling of brains to try to extract general principles,” says Gilles Laurent, a neuroscientist at the Max Planck Institute for Brain Research in Frankfurt, Germany, who has studied locusts, fruit flies, honeybees, zebrafish, rats, cuttlefish and turtles. “Even when you discover differences, after appropriate abstraction, you can discover similarities at the algorithmic level.”
Neural circuits in both pigeons and locusts, for example, process looming stimuli (designed to mimic an approaching predator) in a similar way. “Neural responses can be described by the same mathematical operation, suggesting the existence of converging solutions even though they are implemented in completely different systems,” Laurent says. In locusts, a single neuron performs the computation, whereas pigeons employ a population.
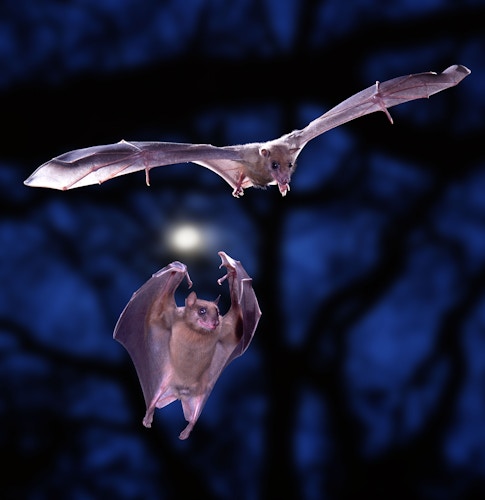
“No one model has it all,” Yartsev says. “The lab mouse is quite different from a human, much like a bat is different than a human and mice. We have to think carefully about what they share, and perhaps more importantly, what they don’t share.”
Egyptian fruit bats:
Ulanovsky and Yartsev’s favored subject is the Egyptian fruit bat, a small species found in Africa and the Middle East. Flying mammals present a particularly interesting species in which to study the function of the hippocampus, a brain area essential for navigation. “We can ask how 3D space is represented in the brain,” says Ulanovsky, who along with Yartsev has developed miniature wireless devices to record brain activity while the animals are in flight.
Neuroscientists have characterized a number of cell types in rodents that are important for navigation, including place cells, which respond to a specific location in the environment, and grid cells, which act as a sort of internal GPS. Yartsev and Ulanovsky have found similar cells in bats. By studying the activity of these cells when animals were in flight, the researchers were able to identify spherical receptive fields. Rodent place cells, by contrast, have only been measured in two dimensions, as animals navigate a maze, for example.
Researchers are now studying the bat equivalent of grid cells. In rodents, grid cells create a 2D hexagonal tiling of space, in which the central point of each hexagon is equidistant to those around it. In research presented at the Cosyne conference in Lisbon in March, Gily Ginosar, a graduate student in Ulanovsky’s lab, searched for a similar structure formed by bats’ 3D grid cells. Using existing models and other information, researchers predicted their grid cell receptive fields would form a 3D lattice — like oranges carefully stacked at the supermarket.
They found that bat grid cells also have a locally fixed distance between neighboring fields. But to their surprise, this local pattern doesn’t create a global grid. In work with Haim Sompolinsky, a theoretical neuroscientist at Harvard University and SCGB investigator, researchers applied a model borrowed from physics (originally developed to explain structure in solids and liquids via pairwise interactions between molecules) to analyze grid cells in two and three dimensions. The hexagonal structure characteristic of rodent grid cells emerges from the 2D version of the model. But in 3D, it produces a structure with local but not global order. “With the extra degree of freedom in three dimensions, you still get fixed local distances, but no global order,” Ulanovsky says.
The finding is useful because the lack of a global lattice rules out some existing models of 2D and 3D grid cells. “This is an example of the benefit of a comparative approach,” Ulanovsky says. “By going to another animal, you can constrain the types of models that can explain the data across species.”
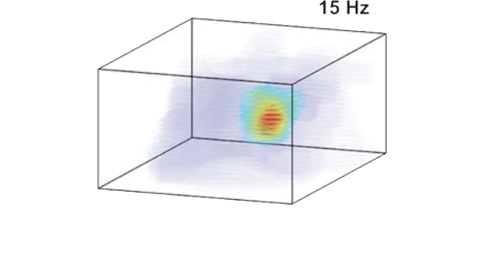
In 2017, Ulanovsky and his collaborators identified an entirely new type of spatial cell in bats — neurons that represent not where the animal is in space, but where it wants to go. Dubbed vector cells, they represent distance and direction to the target location. Models based on data from rodents had predicted the presence of vector cells, but no one had found them before this, Ulanovsky says.
Studies are also helping scientists understand neural coding and navigation at different scales. Egyptian fruit bats have an extensive range, often flying several kilometers from their home cave to fruit trees to forage. Ulanovsky’s group is taking advantage of this range to examine whether the brain employs different mechanisms for mapping large spaces. “We can’t assume that neurons represent space in a 1-meter box the same way they represent a 1-kilometer distance,” he says. Tiling a bat’s trajectory using the 10-centimeter place fields typically measured in rodents would require a quadrillion (1015) neurons, he says. “But the entire dorsal hippocampus has 150,000 neurons.”
Preliminary results from studying place-cell activity as bats fly in a specially constructed 1-kilometer tunnel suggest they have much larger place fields, on the order of 30 meters. Moreover, an individual place cell can have multiple place fields, firing when the animal is in 10 or more different locations. The fields for an individual cell vary in size — in one location it might have a large, 20-meter field, in another, half a meter. “Having one neuron with multiple spatial scales is surprising; not a single model of place cells can explain this, as far as we know,” Ulanovsky says. Researchers are examining how neural circuits might generate this type of flexibility and why it might be helpful to bats.
“Another advantage of studying non-standard species is that it sort of opens your mind,” says Ulanovsky, who spends time with ecologists and ethologists who study bats in the wild. “There is a different community of people studying them; they think differently, which helps you think differently.”
Fruit bats are highly social, often hunting in groups, which researchers are exploiting to examine how the brain acts during social interaction. In a paper published in Cell in June, Yartsev’s team recorded for the first-time neural activity simultaneously from the brains of multiple animals engaged in natural social interactions, such as grooming, fighting or mating. They found that animals interacting with each other showed very high levels of correlated neural activity across their brains. This correlation persisted across a very wide range of timescales which are relevant for social behavior, from seconds to hours, and couldn’t be explained simply by shared movement and sensory inputs.
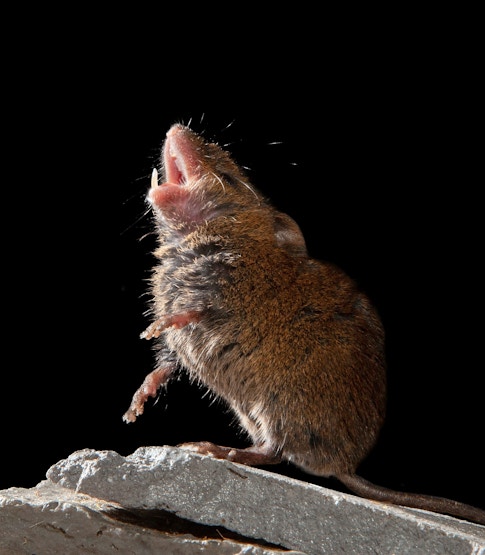
Singing mice:
Speech is challenging to study in animal models, for obvious reasons, so scientists instead focus on specific aspects of vocalization, such as vocal learning. Zebra finches, which learn to sing from their fathers, are the favorite model organism for this pursuit. But being birds, their neural circuitry is far different from our own, limiting how well findings will generalize to humans. New York University’s Michael Long, who studies both birdsong and human speech, sought a mammalian alternative. He settled on the Alston’s singing mouse, Scotinomys teguina, which lives in the cloud forests of Costa Rica and produces stereotyped vocalizations.
Long and his collaborators initially planned to study how these mice learn and produce their elaborate songs. But two years ago, a student in the lab noticed an interesting feature of the animals’ songs — when a mouse hears its neighbor sing, it will start singing in response, sparking a volley of vocalizations that resemble a conversation. Scientists don’t yet know the precise purpose of these vocal exchanges, but it’s likely some kind of territorial display. “As soon as they start singing in tandem, one mouse will often leave its home base and either befriend the other mouse or attack the other mouse,” Long says. “It’s really striking.”
Many species, such as marmosets, call back and forth, but these vocalizations involve long pauses. Human conversation is rapid, with a pause of just one-fifth of a second between speakers, about twice the duration of an eye blink. Singing mice’s exchanges are significant for their speed — they respond to each other almost as rapidly as people do, with a half-second delay between one animal’s turn and the start of the other’s. “We’re looking at the rudiments of a conversational exchange,” Long says.
Long and his collaborators quickly shifted their focus in singing mice from vocal learning to turn-taking and reported their initial exploration of the neural circuits underlying tandem singing in Science in March. They found that a region known as the orofacial motor cortex (OMC) is essential. Silencing this region didn’t stop mice from singing, but they were no longer able to coordinate with their partners. Long and his team determined that these mice possess two song-processing centers. The OMC acts as a sort of conductor, coordinating partners, and a not-yet-identified region generates the song itself. (For more, see ‘Singing Mice Hint at How Sensory Input Turns Into Behavior.’)
Long’s team is now working with SCGB investigator Shaul Druckmann, at Stanford University in California, to better understand the sensorimotor processing — the relationship between one mouse’s song and the other’s motor response — underlying back-and-forth singing. “There are lots of fundamental computations underlying communication, but because mice don’t do it, it simply hadn’t been studied,” Long says. “If we can see the interactions and the neural mechanisms underlying those interactions, I think we can open door to studying human conversation.”
Bats offer another alternative to songbirds for studying vocalization. Bats have an array of communication calls that they emit exclusively during social interaction — these are distinct from echolocation. Unlike zebra finches, the most commonly studied songbird, bats’ vocalizations aren’t stereotyped but instead change according to circumstance.
Yartsev and his team are the first to study the neurobiology of vocal learning in bats, so they first had to map out the brain regions involved in vocal production and interpretation. They identified a region related to the prediction of calls. It is active not just when a call is produced, suggesting it’s involved in higher-level coding — alerting the listener that another bat is communicating. “We think it’s an instructive signal,” Yartsev says. “These are affecting the brain of both the producer and the perceiver.” Researchers are studying neural representations of these different aspects of vocalizations, from early development through adulthood.
Yartsev is careful not to call bats vocal learners, a term he says can be misleading if used too loosely. “We know little about what gives us the vocal-learning capacities we possess as humans,” he says. “To say something informative and potentially useful about humans, we have to be more careful: What precisely do bats learn, what don’t they learn and what do they learn differently from humans?”
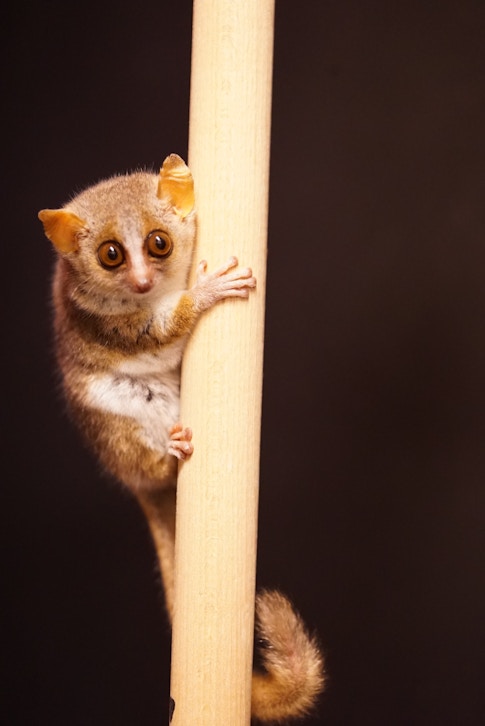
Mouse lemurs:
Daniel Huber, a neuroscientist at the University of Geneva, has been studying lab mice for most of his career. But a new species has recently taken up residence in his lab — the mouse lemur, a tiny nocturnal primate native to Madagascar. Huber aims to take comparative-systems neuroscience to the next level by studying mice and mouse lemurs side by side.
Directly comparing brain activity in rodents with that of the most commonly used lab primates, macaques, is difficult because researchers tend to use different tasks and techniques in each species. But mouse lemurs’ small size — about twice the weight of mouse, with a rat-sized brain — makes it possible to use many of the tools developed for mice, such as calcium imaging, electrodes, behavioral boxes and virtual reality environments, in mouse lemurs with little adaptation. “Because of the mouse lemur’s small size, we can use the entire tool set of systems neuroscience,” says Huber, whose mouse lemur research colony is housed at the National Museum of Natural History in Paris. “All these things make it an extraordinary model system.” Four years in, he says that transferring rodent tools to mouse lemurs has been more successful than he expected.
Huber is closely coordinating his mouse and mouse lemur work to better understand their similarities and differences. “Having both systems in the same labs allows us to level out all these differences — the only difference left should be species-related circuit differences.” Preliminary research presented at Cosyne hints at differences in the visual system. Mouse lemurs have a rodent-sized brain but process visual information like a primate, Huber says. “It’s not just size that matters, but also origin.”
“People who work with mice sometimes don’t step back and say, ‘Is this the right model system to look at?’” Huber says. “All our data with mouse lemurs confirms what macaque data has shown: that there are significant differences when you compare primate and rodent.”
The researchers are working on a high-resolution brain atlas and other resources that they plan to make available to anyone who is interested. “The long-term goal is to get the animal model out there,” Huber says.
One of the mouse’s greatest advantages as a model species is the large number of genetically engineered lines available. Huber is frequently asked whether he plans to make transgenic mouse lemurs. “I don’t think this is necessary anytime soon because we’re using viral and molecular tools that are already working,” he says. “An argument for traditional models is that you can’t do transgenics in other species. But for many of the general principles in systems neuroscience, you don’t really need that many transgenic tools.” Moreover, gene-editing tools, such as CRISPR, are making it easier to genetically modify a variety of organisms.
Huber and others’ forays into a broader world of animals may mark a new trend in the field. After a period of constriction, Ulanovsky thinks neuroscience’s choice of animal models is starting to widen again. “It makes sense for standard models to be the majority,” he says. “But the neuroscience community should strive for nonstandard models being a larger minority, 10 to 15 percent rather than 1 percent.”
That expansion needs to happen soon, Laurent cautions. “Culture and knowledge of a variety of model systems is disappearing,” he says.