Technology Mashup Images Single Cells and Whole Brain Areas
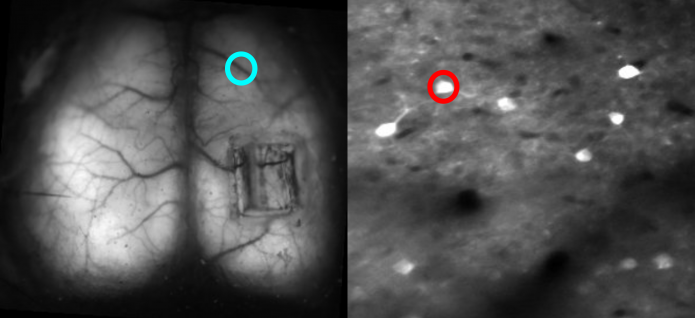
A central focus of the BRAIN Initiative (Brain Research through Advancing Innovative Neurotechnologies), a federal neuroscience grant program launched in 2013, is the development of new technologies to explore the brain on different levels simultaneously — by examining individual cells and larger circuits, for example, or both electrical and chemical activity.
Several novel technologies are already emerging from the program, including a microscope designed to capture wide-field and two-photon imaging at the same time and a minimally invasive electrode array that can record both spikes and dopamine levels. Both were presented at the BRAIN symposium at the Society for Neuroscience conference in Washington, D.C., in November 2017.
Inspired by a call for multiscale-imaging proposals, Michael Crair, a neuroscientist at Yale University, began to think about how to bring together two fundamentally incompatible modalities — wide-field and two-photon imaging. Wide-field (or one-photon) microscopy captures the neural activity of a large swath of brain tissue. Two-photon microscopy images smaller segments at high resolution, down to the level of single cells. Fusing the two enables neuroscientists to explore how the activity of individual neurons relates to activity across the brain.
The main challenge is that the two approaches require different optics. The excitation light for wide-field microscopy disrupts two-photon imaging, and vice versa, “so you need to physically and optically separate them in order to put them together,” Crair says.
Dan Barson, a graduate student at Yale, developed a device with Crair and collaborator Michael Higley in which the two-photon lens is rotated 90 degrees with respect to the wide-field lens — the latter images from the top of the animal’s head, while the former images from the side. A microprism above the brain bounces light at a right angle so that the fluorescence from individual cells can be collected by the two-photon objective lens. The microprism is small enough — 1 to 2 microns square — that it doesn’t impact wide-field imaging.
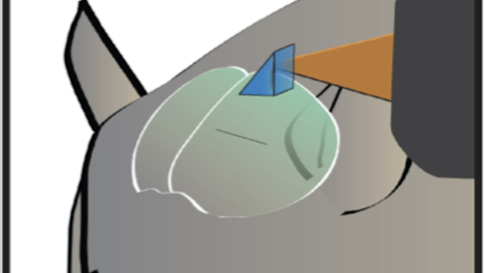
To solve the optical conflict, researchers interleave wide-field and two-photon imaging. “It’s essentially like imaging every other frame, fast enough so that it’s virtually simultaneous,” Crair says. They then separate out the frames and recombine them.
The two-photon component is not as good as the best two-photon devices because the microprism limits resolution. But the lens can detect single cell activity. “None of these things in and of themselves are heroic or revolutionary in terms of optics,” Crair says. “It’s partly thinking through the problem and coming up with imaginative solutions.”
So far, the researchers have used the device to measure broad cortical activity and local activity in hundreds of cells, tracking ongoing dynamics in different cortical areas and the relationship between single-cell and wide-field activity. Some cells are tightly correlated to the contralateral cortex, others to the motor cortex.
The researchers are now using the technology to study functional connectivity in the developing brain. Scientist have traditionally used fMRI, and more recently wide-field imaging, to determine when two brain areas are functionally connected, meaning their activity rises and falls together. But simultaneous wide-field and two-photon imaging makes it possible to examine functional connectivity at a finer scale. For example, scientists can determine whether different neurons within the same region connect to different places. “By adding in two-photon imaging, we can figure out which classes of neurons [within a brain region] are responsible for functional connectivity,” Crair says.
Researchers can also look at whether connectivity changes over time or differs between cortical layers or brain regions. Studies employing fMRI suggest that functional connectivity morphs with the task. “We are in a position now to not only measure the pattern of connectivity but to look at when those connectivity matrices change,” Crair says. “Does behavior change which cells are connected or does it change their activity? It’s plausible that local networks change the structure, which changes global functional connectivity. Or maybe some cells stop being active and others start. We can check for that.”
The researchers hope that other groups will employ the technology as well. “Our feeling that anyone with some experience in two-photon could set this up in their own labs without too much difficulty,” Barson says.
Other researchers are developing new electrode microarrays for directly recording neurons’ electrical activity. One of the problems with traditional arrays is the large size of the electrodes, which damage surrounding tissue when inserted. This limits recording duration and damages the circuits they record from. Cynthia Chestek, a chemical engineer at the University of Michigan in Ann Arbor, is developing high-density carbon fiber arrays with hair-thin electrodes smaller than the width of a neuron. The tiny size dramatically reduces tissue damage at the recording site. “Our electrodes are like cobwebs,” Chestek says.
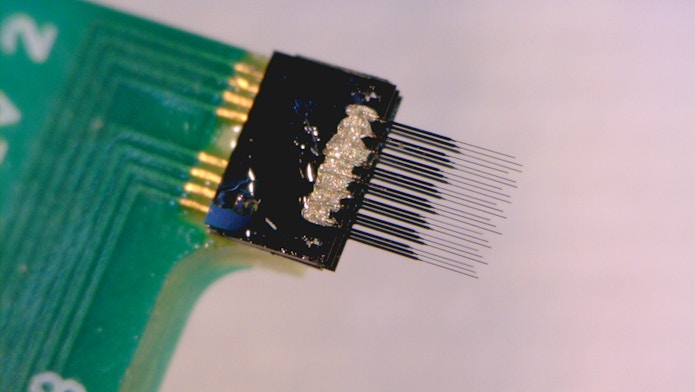
Chestek and collaborators published details of the technology in 2016, showing it can record neural activity for months with minimal scarring of the tissue. The team is now developing a method for slicing the brain with the electrodes still in place. “To our knowledge, this may be the first direct visualization of neurons adjacent to an electrode that was recording them,” Chestek says. Preliminary results show that neurons around the electrode look normal, confirming that the device causes minimal damage. “These seem to be invisible to the brain,” she says. Ultimately, researchers want to visualize the neurons that they recorded from and then map the broader neural circuit.
“Chestek’s group has come up with innovative methods for implanting carbon fiber electrodes with tiny footprints and demonstrated recording of single-unit activity up to four months,” says Duygu Kuzum, a bioengineer at the University of California, San Diego. “Their work on stable carbon fiber microelectrode arrays is a big step towards achieving chronic brain interfaces with long-term stability.”
Chestek’s approach is very different from another new recording technology, Neuropixels, which look like long needles tiled with hundreds of recording sites. Neuropixels probes are inserted deep in the brain and can record from many neurons in a narrow column of brain tissue. The electrodes in Chestek’s array, in contrast, are spaced about 100 microns apart, covering a large segment of cortex but with a shallower reach.
The flexibility of carbon fiber electrodes makes it difficult to insert them deep into the brain. Researchers can access layer five of the motor cortex, about 500 to 1,500 microns deep, with naked carbon fibers. But reaching deeper subcortical areas requires a silicon support structure, which can cause tissue damage.
The carbon fiber composition offers an advantage, however. Researchers can co-opt an existing method, known as fast-scan cyclic voltammetry, to track dopamine. Chestek and collaborators use cyclic voltage to oxidize dopamine on the electrode and measure the resulting current, which is proportional to dopamine concentration around the electrode. The dopamine-tracking devices are slightly different from the original arrays but can still record single-cell electrophysiology, though not as efficiently as devices optimized for that purpose. “Right now, we have to optimize for one or the other,” Chestek says.
Chestek’s team is now expanding the project, working with beta testers and looking for funding to help optimize and distribute the device. “I would love to hear from people who want to use it,” she says.