Visualizing Physics at the Nano-Scale
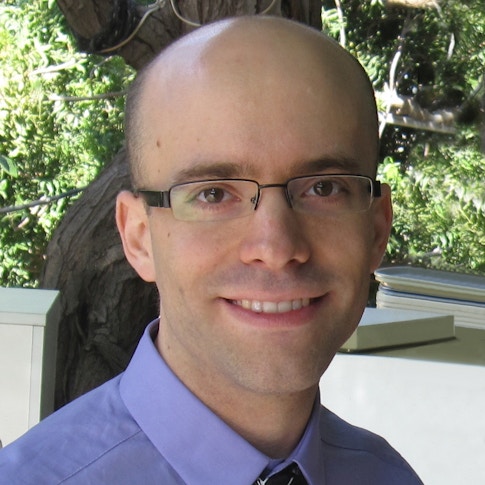
When Dorri Halbertal decided early in his career to leave his chosen field of theoretical astrophysics, he sought something “more down to Earth — literally.” He settled on experimental condensed matter physics, an area of research that seeks to understand at the quantum level the properties of materials — anything from ordinary solids and liquids to more exotic phases of matter like superconductivity. “Part of what drove me to transition from theory to experiments is finding impact in the real world,” he says. “You cannot cheat nature. It either works or not. So, when you have an idea, you can actually test it and see whether you have any grasp on it in reality or if it’s just a fiction.”
Now a postdoctoral fellow at Columbia University, Halbertal is focusing his research on using novel methods to visualize nanoscale physical processes that would typically be invisible to microscopy — like the way that electrons are scattered by a single misaligned atom in a sheet of graphene. “There are some more commonly used techniques which are very powerful,” he says, “but I find the road less taken more fitting.” An edited version of my interview with him follows.
Your early work in astrophysics focused on simulating supernovae. What did you discover?
Back then, I was trying to quantitatively simulate how radiation drives a shock wave in a supernova. There are details that are hard to capture in a true calculation due to the multiple length-scales and energy-scales that the problem involves. It was done previously in very approximate ways by making some crude assumptions, which led to oversimplified solutions. It’s actually more complex, the way that radiation is involved in actually driving the explosion of the star. I was able to show that something was missing in these [previous] descriptions.
After success early in your career, what made you want to switch fields?
My master’s thesis adviser also asked me that: “This is the moment to reap the fruit of your hard work; this is actually when you can benefit from all of the effort that you’ve invested!” But something was missing for me. It was just me and the computer. I enjoyed part of it, but I wanted the work to have some more realistic aspect.
Then you moved into condensed matter physics. Why?
Condensed matter physics is dealing with complex interactions between electrons in the matter. For instance, if you cool down a material, at some point it sharply changes its behavior, becoming a perfect electrical conductor. The electrons behave as if they were condensed — as if they were part of a common liquid instead of individual particles — and they all move together synchronously.
I find these correlated behaviors almost magical. What would cause an ensemble of 1023 electrons to start behaving as one? I’m interested in giving a very intimate view of what is happening locally in these systems.
Your work employs ‘optical’ methods for investigating these phenomena. What do you mean by that?
It’s not ‘seeing’ as you would think, like when you put a certain sample under a microscope and just have a look. It’s subtler than that, because there is a fundamental problem with using light in order to probe physics on this scale: the diffraction limit. This limit means that the wavelength of light you’re using to see a feature can’t be longer than that feature — otherwise the image will just be a blur. Moreover, sometimes we are interested to ‘see’ the material using other ‘lenses,’ which do not necessarily involve light at all. For instance, in my Ph.D. work I used a special local probe called a nano-SQUID [superconducting quantum interference device], which is sensitive to small changes in the local magnetic field. But I used it in order to detect very small changes in heat dissipation. Using such an approach, we were able to track such small changes in the local temperature that it led us to introduce a new concept of thermal imaging of quantum materials. We used that technique to track how electrons lose their energy when they are injected into a graphene chamber. They bounce back and forth like billiard balls until they encounter an atomic scale trap on the edges, and this allows them to transfer their energy to the graphene lattice as a vibration.
Nowadays, I am actually using light to study materials, but in an unconventional way. We bypass the diffraction limit by using a very sharp object, like a metallic tip, as a probe, and focusing light on the tip. Basically, we use this tip as an antenna that locally interacts with the material at hand: In one case we can measure the light that is scattered as a result of this interaction, and in another case we can directly probe the electrons. The tip as an antenna causes electrons to run around and bounce around, and then heat is produced. So, locally there’s a ‘hot spot‘ under the tip, which we measure with electrodes. In both cases, although the wavelength of light that we use is very large compared to the features that we are studying, because the hot spot is localized, we’re able to resolve features which are way, way smaller than the diffraction limit. Scanning the features with this tip gives you a way to optically study the nanoscale.
Why is this kind of investigation worth doing, if other techniques for probing the nanoscale already exist?
Whenever you go to higher and higher resolution in images, there’s always new insights involved. An image is worth a thousand words — probably even more so in science, because you often have a certain, very oversimplified picture in your mind of what is happening. But if you have the right tool to actually visualize it, this is the ultimate test of a theory.
What have you been able to see with this technique?
Let’s say you take two layers of graphene, which is a sheet of carbon atoms, one atom thick. And instead of just stacking them one on top of the other, you introduce a very small but controlled twist between them. You can think of it as placing one net on top of another, with a slight twist. It creates a visual pattern called a moiré effect. And this moiré effect creates a perturbation to the energy landscape of the graphene, with some quite remarkable features. And I’m interested in visualizing the different properties that emerge in such a structure.
Have there been any unique challenges in your career, given the sharp turn it took from one field to another?
Making a sharp change is always hard. I think that my philosophy in general is not to stay in my comfort zone. So far, it has been quite an interesting journey for me each time, to learn from scratch, to be a novice. I did take a gamble, I suppose, in the transition I’ve made. But I tend to get to some interesting places.